The Big Bang’s mysteries and unsolvable “first cause” problem
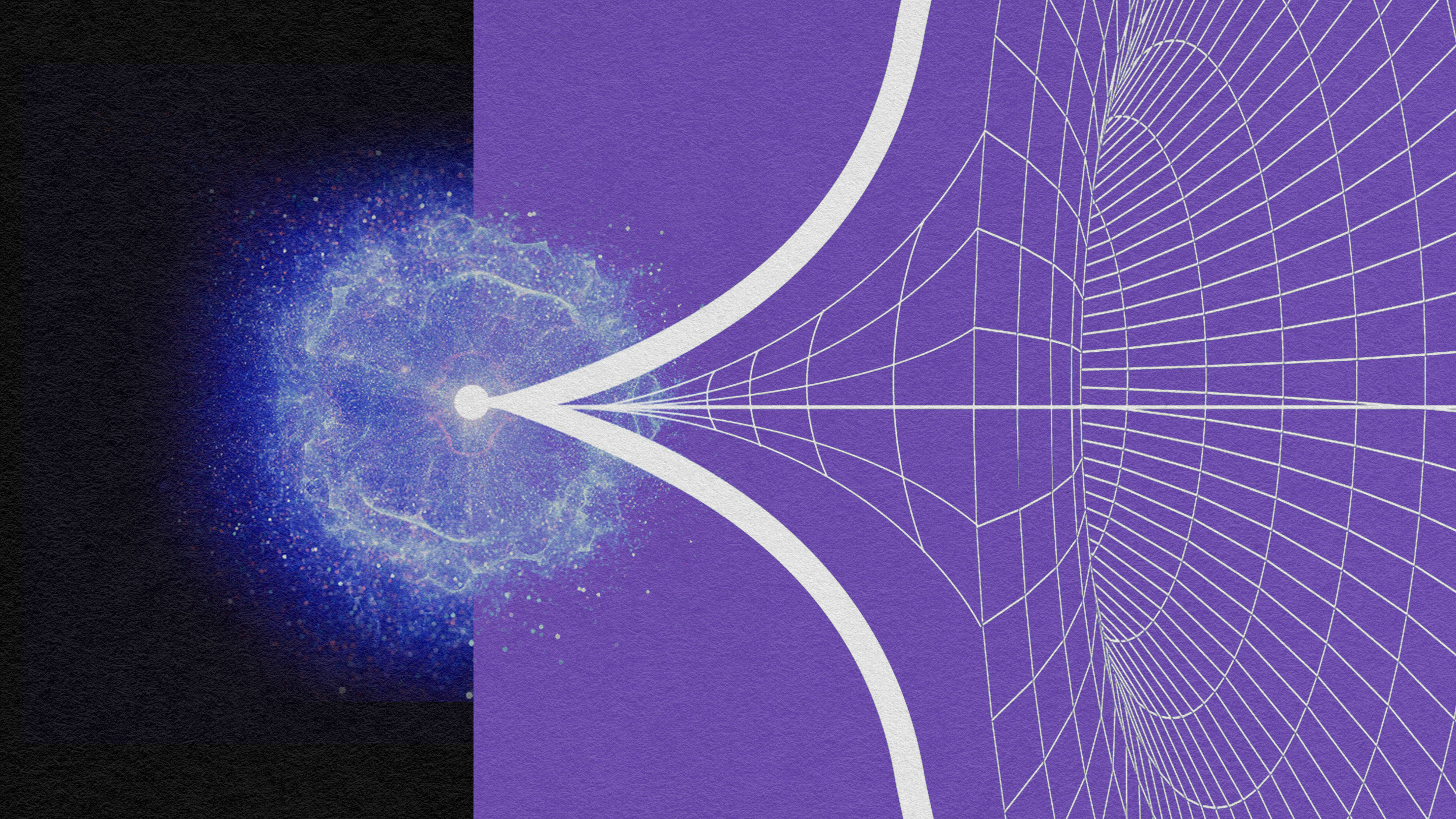
- The quest to understand the Universe’s origin has evolved from mythic narratives to the quantitative insights of modern cosmology, sparked by Einstein’s theory of relativity and its implications for understanding the cosmos’s structure and expansion.
- Key discoveries, such as the expanding Universe through Hubble’s observations and the Big Bang theory’s predictive success, have grounded our cosmic understanding in observable phenomena, revealing a Universe that was once hotter, denser, and more uniform.
- Despite significant advances, the earliest moments and the fundamental cause of the Universe’s inception remain shrouded in mystery — perhaps forever so.
If there’s one question that has been present throughout human history across all cultures, it’s the question of the origin of all things. Why is there a Universe? How come we exist in it to be able to ask this question? Across millennia, different cultures offered mythic narratives to address the mystery of existence. But with the development of modern science, the focus has shifted to a more quantitative approach — a scientific narrative of the origin and history of the Universe, the focus of modern cosmology.
It all started in 1915 when Albert Einstein proposed his new theory of gravity, the general theory of relativity. Einstein’s brilliant innovation was to treat gravity not as a force acting at a distance, as did Newton, but as the curvature of space due to the presence of mass. Thus, according to Einstein, the orbital motions of celestial objects are caused by the spatial curvature of their surroundings. A way of visualizing this is by throwing marbles across a mattress. If no weight bends the mattress, the marbles will move along straight lines. But if you place a heavy lead ball on the mattress, the marbles that roll nearby will trace curved paths. If you practice your throws, you can get the marbles to circle the lead ball, somewhat like planets circle the Sun. Einstein’s theory allows physicists to calculate the geometry of the bent space around an object. He demonstrated his theory’s validity by both showing how Mercury’s orbit wobbles about the Sun (the precession of Mercury’s perihelium) and by computing how starlight gets bent as it travels near the Sun.
Two years after he launched his new theory, Einstein took a bold step, moving from solar system applications to the whole Universe. He figured that he could solve his equations to estimate the geometry of the Universe as it is bent by the matter inside. To do this, he made three simplifying assumptions: that the Universe is spherical, that it is static, and that matter is, on average, distributed equally everywhere in space (this latter assumption became known as the “cosmological principle”). To his surprise, his imaginary Universe wasn’t stable: perturb it a bit and the whole thing collapses into a point due to its own gravity. Disappointed but not defeated, Einstein added an extra term to his equations to act as a counterbalance to gravity’s attraction: the so-called “cosmological constant.” Adjusting its value, Einstein managed to find his static spherical solution. Hence modern cosmology was born.
Einstein’s pioneering work inspired many theoretical physicists to build their own desktop Universes — mathematical models that changed his assumptions to see what would happen. In 1917, the same year of Einstein’s model, the Dutch Willem De Sitter solved the equations for an empty Universe (one with no matter at all) with the cosmological constant. His solution, not surprisingly, described a space where two points would exponentially move away from one another. In 1922, the Russian Alexander Friedmann abandoned Einstein’s assumption of a static Universe and found, to his delight, solutions for a Universe that would grow with time. How fast it would grow depended on the value of the cosmological constant and on the type of matter that filled all of space.
While theoreticians were imagining different desktop Universes, the American astronomer Edwin Hubble was pointing the 100-inch telescope at Mount Wilson to determine whether the Milky Way was the only galaxy in the Universe or whether there were many galaxies out there — “island Universes” spread across space. In 1924, 100 years ago this year, he hit on the solution: The Milky Way is but one of billions of galaxies out there. The Universe suddenly became enormous, beyond what we humans could contemplate. Five years later, Hubble dropped the real bomb: Not only were there countless galaxies in the cosmos, but the vast majority were moving away from one another. The Universe, Hubble concluded, is expanding. This remarkable discovery changed everything. If the Universe is growing in volume and galaxies are moving apart, that means that in the past they were closer together. Using some coarse approximations, Hubble estimated that some 2 billion years back, galaxies would all be squeezed into a very tiny volume. This would represent the beginning of the comic history. The Universe, it turns out, had a beginning at some distant point in the past.
The Big Bang model
In the late 1940s, the Russian American physicist George Gamow worked with Ralph Alpher and Robert Herman to figure out what could be the story of an expanding Universe. The essential point is that if the Universe was expanding now, then in the past it was smaller, hotter, and denser. Before galaxies, stars, and planets existed, matter was crunched up and broken down into its most basic constituents. Mixing atomic and nuclear physics, the trio arrived at a few stunning conclusions. The first was that a Universe originating in a hot, dense state should now be suffused with microwave radiation, a relic from the era of recombination when hydrogen atoms first formed as protons and electrons joined, thereby releasing photons to traverse the cosmos freely. In modern numbers, that happened about 380,000 years after the Big Bang, the event that marked the beginning of time, the time that clocks the expansion of the Universe. In 1965, the radiation predicted by Gamow, Alpher, and Herman was discovered by Robert Wilson and Arnold Penzias, two radio astronomers working for Bell Labs in New Jersey.
The second prediction from Gamow, Alpher, and Herman was that going even further back in time, the Universe would be so hot and dense that protons and neutrons would roam free, unable to combine to form the first atomic nuclei. As recounted in Steven Weinberg’s classic book The First Three Minutes, the synthesis of the lightest nuclei — or primordial nucleosynthesis — happened when the Universe was about one second to three minutes old, as protons and neutrons combined to form deuterium and tritium, isotopes of hydrogen with one and two neutrons attached to the proton, respectively, and also helium-4 (two protons and two neutrons) and its isotope helium-3 (two protons and one neutron), and, finally, lithium-7 (three protons and four neutrons). Combining the rules of nuclear physics with the thermodynamics of an expanding and cooling Universe, it was possible to estimate the abundances of these elements and compare them to observations. The concordance was another great success for the Big Bang model. By the late 1960s, there was no doubt that the narrative of a Universe that started hot and dense and with matter dissociated into its simplest constituents correctly described the cosmic infancy. The question in everyone’s minds then was: What about earlier times? How far closer to the beginning of time could physics get? This is where things start to get both more exciting — and murkier.
Toward the beginning of time
As we try to push the reach of our theories to earlier times, we move up in energy from atomic and nuclear physics to particle physics. After all, the earlier you look at the Universe, the hotter and denser it was, and so the particles that filled up space had higher energies. Thus, to dive deeply into the cosmic infancy, physicists must use concepts from high-energy physics, going beyond experimental results. With some confidence, we could push the clock back to one-hundred-thousandth of a second after the bang, when the Universe had energies comparable to those when protons and neutrons got broken down into a quark-gluon plasma, a state of matter that has been studied in the past two decades or so with great success but also with some serious conceptual limitations. Still, we have good reason to believe that this state actually existed in the early Universe, presenting a wall behind which matter was dissociated into its simplest known constituents. That is, before this, we can really talk about a primordial soup of elementary particles filling up space.
The spectacular discovery of the Higgs boson in 2012 confirmed that we could study different forces of nature under the same framework. We know of four fundamental forces: the gravitational, the electromagnetic, and the strong and weak nuclear forces. The two latter forces are only active at subnuclear distances, explaining why we are not familiar with them in our everyday lives. What the discovery of the Higgs confirmed was that the electromagnetic and weak forces tend to behave in similar ways at very high energies, at the limit of what our current experiments can probe. If we map these energies to the early Universe, we are talking about one-trillionth of a second after the bang, or 10-12 seconds. We don’t understand exactly how particles interact at these energies, but what we do understand, summarized in the Standard Model of particle Physics, indicates that the whole Universe went through a sort of phase transition. This transition resembles the process where cooling liquid water crystallizes into ice, breaking the fluid’s uniform symmetry—where molecules are evenly dispersed—into a rigid, ordered lattice. Similarly, we say that the Universe went through a phase transition at about this early time when the “electroweak” force split into the electromagnetic and the weak forces. Details are missing but that’s the overall picture.
At this juncture, cosmology hits a conceptual barrier, as it needs to navigate backward in time without experimental guidance. Despite decades of efforts across the globe, we have not gathered any information from the very early Universe that could guide us. The solution, of course, is to extrapolate and propose models of the early Universe that are compelling for different reasons. For example, they may offer explanations to current challenges to the Big Bang model, as in the inflationary model; they may open new avenues for research in very high energy physics — as in theories of quantum gravity; or they may inspire experimental work in novel directions — as in, for instance, searches for dark matter and primordial gravitational waves.
Inflationary models were proposed during the early 1980s as potential solutions to several issues plaguing the Standard Big Bang model. For example, observations tell us that the geometry of space is very nearly flat, and we don’t know why. We also don’t know why the temperature of the microwave background mentioned above is so homogeneous, to one part in one hundred thousand. We also don’t know how the matter clumping needed to form galaxies and large cosmic structures originated. Somehow, matter gathered in various spots and attracted more matter. Inflation was proposed originally by MIT’s Alan Guth to address these issues. To do so, it invokes a sort of Higgs-like field assumed to be part of some unknown particle physics model describing the physics of the very early Universe. In the same way that the Higgs field drove the split between the electromagnetic and weak forces at 10-12 sec, the “inflaton field” drove the dynamics of the very early Universe at…10-35 sec. That’s a lot of zeroes into the unknown.
Guth’s model, along with the many alternatives proposed since his pioneering work, rely on this extrapolation, assuming that a primordial field caused the Universe to expand exponentially fast for a very short period of time. As the field loses energy, it relaxes to its lowest energy state and decays into a plethora of particles with explosive effectiveness, causing an ultrafast heating of the Universe. Some cosmologists call this the real Big Bang, though this is a matter of taste. One of the challenges here is to uncover an even earlier history of the Universe, one that actually determines what kind of field this hypothetical inflation was and where it came from. Compelling as cosmic inflation is, we don’t really have either a believable model nor any evidence that it truly happened, apart from concordance with current observations. A handful of models nicely describe the Universe that we see (flat, homogeneous, crumpled up) but we still need a mother theory to give it more fundamental validity. What this theory might be requires even more adventurous extrapolations.
Even if we assume that we can push back our models even further in time, we soon hit a monumental conceptual barrier. As we approach the highest energies that we can still make sense of, the Universe may require a different way to be described, borrowing from quantum physics: the physics of the very small. The point is that in the quantum world, everything is jittery and everything fluctuates. If we carry this notion into the force of gravity — and its relation to space and time — we need to consider the possibility that at very early times, there was no time and space as we know them but some sort of vague quantum foam, where space and time bubbled in different ways here and there (though “here” and “there” become very murky ideas). Unfortunately, our current attempts to describe such quantum spacetime foam in theories known as string theories and loop quantum gravity have been only partially successful or not at all successful, at least in providing a compelling scenario for the origin of the Universe. We seem to be fundamentally stuck when it comes to the question of the origin of all things.
The problem of the first cause
And this is not surprising, once we pay attention to the history of philosophy and the nature of science. The origin of the Universe pushes the boundaries of what we can understand. Simply put, most of science is based on two things: objectivity and causality. Objectivity asks for a clear separation between the observer and what is being observed. Causality assumes an ordering in time whereby an effect is preceded by a cause. As I pointed out in a recent book with my colleagues Adam Frank and Evan Thompson, the origin of the Universe brings both causality and objectivity to a halt. And it does so in a very different way from quantum physics, where both principles are also challenged. Quantum mechanics blurs the separation between observer and observed and substitutes deterministic evolution by probabilistic inference. It is, however, still a causal theory since an electron will respond to, say, an electromagnetic force in ways dictated by well-known dynamical causes (with, for a technical example, a Coulomb potential in Schrödinger’s equation). There are known forces at play that will induce specific dynamical behaviors.
But when it comes to the origin of the Universe, we don’t know what forces are at play. We actually can’t know, since to know such force (or better, such fields and their interactions) would necessitate knowledge of the initial state of the Universe. And how could we possibly glean information from such a state in some uncontroversial way? In more prosaic terms, it would mean that we could know what the Universe was like as it came into existence. This would require a god’s eye view of the initial state of the Universe, a kind of objective separation between us and the proto-Universe that is about to become the Universe we live in. It would mean we had a complete knowledge of all the physical forces in the Universe, a final theory of everything. But how could we ever know if what we call the theory of everything is a complete description of all that exists? We couldn’t, as this would assume we know all of physical reality, which is an impossibility. There could always be another force of nature, lurking in the shadows of our ignorance.
At the origin of the Universe, the very notion of cause and objectivity get entangled into a single unknowable, since we can’t possibly know the initial state of the Universe. We can, of course, construct models and test them against what we can measure of the Universe. But concordance is not a criterion for certainty. Different models may lead to the same concordance — the Universe we see — but we wouldn’t be able to distinguish between them since they come from an unknowable initial state. The first cause — the cause that must be uncaused and that unleashed all other causes — lies beyond the reach of scientific methodology as we know it. This doesn’t mean that we must invoke supernatural causes to fill the gap of our ignorance. A supernatural cause doesn’t explain in the way that scientific theories do; supernatural divine intervention is based on faith and not on data. It’s a personal choice, not a scientific one. It only helps those who believe.
Still, through a sequence of spectacular scientific discoveries, we have pieced together a cosmic history of exquisite detail and complexity. There are still many open gaps in our knowledge, and we shouldn’t expect otherwise. The next decades will see us making great progress in understanding many of the open cosmological questions of our time, such as the nature of dark matter and dark energy, and whether gravitational waves can tell us more about primordial inflation. But the problem of the first cause will remain open, as it doesn’t fit with the way we do science. This fact must, as Einstein wisely remarked, “fill a thinking person with a feeling of humility.” Not all questions need to be answered to be meaningful.